Abstract
The substitution of fossil fuel power plants with renewable units will lead to a profound reduction of CO2 emissions and will assist in evading the Global Climate Change. Using demand data from the electricity grid of Texas, this paper develops a scenario for the substitution of coal, at first, and of all fossil fuel power plants, secondly, in the entire State of Texas. Meeting the electricity demand of the grid with renewables, makes it necessary to develop significant energy storage capacity in addition to the renewable – wind and solar – installations. Because of the lower pant capacity factors of wind and solar units, the calculations show that significantly higher renewable power capacity must be built than the current capacity of the fossil fuel units to be substituted. Also, that a substantial fraction of the generated energy is lost in the storage-recovery processes. All these factors are expected to increase the price of electricity paid by the consumers. Ahis will have an impact on the less affluent segments of the population and – if ignored by national energy policies – will affect the goal to reduce inequality within and among countries.
License
This is an open access article distributed under the Creative Commons Attribution License which permits unrestricted use, distribution, and reproduction in any medium, provided the original work is properly cited.
Article Type: Research Article
EUR J SUSTAIN DEV RES, Volume 4, Issue 1, 2020, Article No: em0111
https://doi.org/10.29333/ejosdr/6344
Publication date: 07 Dec 2019
Article Views: 4193
Article Downloads: 2190
Open Access HTML Content References How to cite this articleHTML Content
INTRODUCTION
As the global society becomes more industrialized and urbanized, the demand for electric power grows substantially faster than other types of energy. In the period 1978 to 2017 the annual growth of electricity demand was 4.9%, while that the Total Primary Energy Supply (TPES) growth was 1.9%. In the second decade of the 21st century the global electricity demand doubles every 14.5 years and all indications are that it will continue increasing with a similar rate until 2050 (International Energy Agency, 2018; US-Energy Information Administration, 2016; Michaelides, 2018). A significant contribution to the strong growth of the global demand for electric power and the global trends of power demand is a consequence of the increasing use of air-conditioning (a/c) systems during the summer season (Worland, 2018). With more than 81% of the global population residing in the geographical zone between the two 45o latitudes (north and south) where a/c is desirable or essential in the summer, the economic development of several nations in this geographic zone has also ushered the accelerated use of a/c and the significant electricity consumption during the summer months.
Approximately 39% of the global electric energy production is generated from coal and another 23% from natural gas, with the contribution of coal continuously increasing since the beginning of the 21st century (International Energy Agency, 2018). The combustion of the two fossil fuels – and especially the combustion of coal – emits large quantities of CO2 in the atmosphere. CO2 is the most abundant Greenhouse Gas (GHG), and the principal contributor to the average global temperature rise and the Global Climate Change (GCC). Coal power plants produce approximately 1.15 tons of CO2 per MWh and natural gas turbines produce (on the average) approximately 0.75 tons of CO2 per MWh (Michaelides, 2018). The global electric power generation industry contributed approximately 40% of the 38 billion tons of CO2 that was produced in 2016 with all the emissions being point-source emissions (International Energy Agency, 2018; US-Energy Information Administration, 2016). It becomes apparent that the most effective way to reduce the global GHG emissions and elude the GCC is the substitution of fossil fuel power plants – starting with the more offensive coal power plants – with renewable energy sources, primarily with wind and solar energy, which are available in all the regions of the globe. Such initiatives to reduce the CO2 emissions by curtailing the use of fossil fuels for the generation of electricity have been adopted in several regions of the globe, and especially within the European Union.
Increasing the contribution of nuclear energy – a largely non-CO2 emitting energy source – in the mix of electric power generation is another option. However, because of problems associated with the disposal and long-term custody of nuclear wastes, there are very few initiatives around the world to “go nuclear.” Hydroelectric power and geothermal energy are clean and effective solutions, but not available in all the regions of the globe. In addition, most of the prime hydroelectric and geothermal resources have been already utilized (Michaelides, 2016, 2018) for the generation of electricity in the OECD and most developing countries. This leaves solar and wind power – two abundant but diffuse energy sources – as the principal alternatives for the substitution of fossil fuel power plants.
The main principle of sustainable development is the ability of a society to ensure that it meets the needs for the present, without compromising the ability of future generations to meet their own needs (Brundtland, 1987). This general principle, which was coined by the Brundtland Commission, has generated sets of guidelines for nations to achieve higher standards of living (development), while taking under consideration the interrelationships between people, resources, and the natural environment. Guidelines for sustainable development recognize that economic development is what humans do as they attempt to improve their conditions within their habitat; and that the environmental, social, and economic factors, which affect all nations and human societies, are highly interconnected and inseparable.
The economic development of nations has always been accompanied by the significant increase of energy consumption and, especially, of electricity. Table 1 shows the increase of the GDP of the entire world as well as of five nations in the period 2001 to 2016, adjusted for purchasing power parity (PPP); the corresponding TPES growth; and the electric energy production growth in the same time period (International Energy Agency, 2003, 2018).
Table 1. GDP (adjusted for PPP), TPES and electricity consumption of the world and in five nations, in the years 2001 to 2016. Data from International Energy Agency (2018) and International Energy Agency (2003)
|
Two significant trends are apparent in the data of Table 1:
-
The economic development of nations (GDP growth) is accompanied by significant growth in TPES consumption and electric energy generation.
-
The rate of electric energy consumption growth significantly surpasses that of TPES. This trend extends to most nations during the period of the first two decades of the 21st century.
It must be noted that one of the unintended consequences of higher electricity generation in the period 2001-2016 is the increased combustion of coal, which was the primary contributing factor to the global CO2 emissions growth – from 25,406 billion tons of CO2 in 2001 to 38,100 billion tons of CO2 in 2018 (Boden et al., 2012; Michaelides, 2018).
Electricity is transmitted from the generating power plants to the consumers via a vast network of transformers and cables that are parts of the regional electricity grids. These grids do not store electric energy. At any instant, the consumers’ demand for electric power is matched by the electricity supply from the generating stations. Of the latter, the very large base-load units primarily use Rankine cycles with nuclear or coal as their fuels; intermediate- and peak-load power plants essentially operate with Brayton and Diesel cycles (or with combined cycles) and use gaseous hydrocarbons or liquid petroleum products. These power plants are available to produce power at will and their operation closely follows the regional power demand.1
The vast majority of power plants operating with Rankine cycles are base-load units that cannot be switched on and off frequently. Power generation in such units may be slightly reduced – to approximately 80% of their rated capacity by steam throttling. Frequent, large scale power fluctuations and stoppages are avoided in these power plants because they damage the machinery. Nuclear and coal-fired steam power plants are base-load units that are placed in the grid for almost continuous operation. The diurnal fluctuations of electric power demand are primarily met with gas turbines and, wherever available, with hydroelectric units (El-Wakil, 1984; Michaelides, 2018).
This paper aims at communicating some of the important consequences of fossil fuel power plant substitution with wind and solar units. The paper offers insights on the demand-supply mismatch; the need for energy storage; the evolution of the so called “duck demand curve;” the effects of adding nuclear capacity to balance the required energy storage and plant capacity; and the effect of fossil fuel substitution on the price of electricity and four sustainability goals. While particular reference to the ERCOT electricity grid in Texas is made (for which a great deal of data are available and which is typical of a grid in a region with high a/c utilization), the conclusions of the paper are general and may be extended to most electric power grids where substitution with renewable electricity sources is anticipated.
Demand and Supply Mismatch
Solar and wind are abundant and environmentally friendly forms of energy, but they are very diffuse and not always available when electric power is demanded. Solar is periodically variable and wind power is almost intermittent. As a consequence, the substitution of electricity generated by fossil fuels with the renewable wind and solar energy sources encounters important limitations that are chiefly characterized by supply and demand mismatch.
The first limitation is inadequate supply: During the early evening hours of a hot summer day, when there is widespread use of the a/c systems in most equatorial and temperate regions of the globe, there is only very low solar irradiance and the feeble wind currents are not sufficient to satisfy the (still high) electricity demand. The second limitation is related to oversupply: It is axiomatic that photovoltaic (PV) and thermal solar installations only produce electric power during the daylight hours. When the region of an electricity grid produces a high fraction of its total annual electric energy by solar installations, there is high solar electricity generation during the morning hours. The data on the demand side show that, during the early daylight hours (from the early morning until noon), the demand for electricity is lower because the ambient temperature is not high enough for the a/c units to operate continuously. The high power production from the solar systems in combination with the moderate electricity demand by the households, causes a supply-demand mismatch, which is manifested in the so called U-shaped demand curve, or “duck curve” (Freeman et al., 2016; Weber, 2016). The mismatch becomes significant and affects the entire electricity grid, when the penetration of PV-generated energy in the electricity market exceeds approximately 30% (Leonard et al., 2020). The non-renewable demand reduction levels are significant to negatively affect the operation of the base-load units that utilize Rankine cycles and are designed to continuously produce electric power.
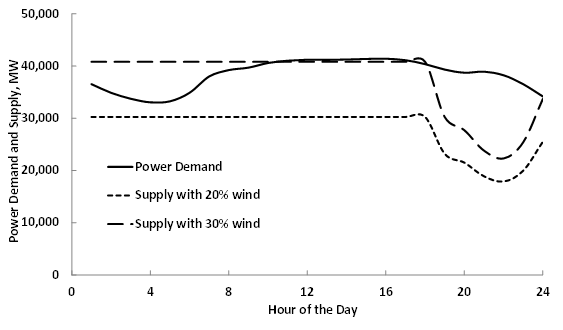
Figure 1 shows this demand-supply mismatch when a relatively large fraction of the annual electric energy is supplied by wind and solar power in ERCOT2 the independent electricity grid that supplies most of Texas (ERCOT, 2018). Figure 1a shows the power demand in this grid on May 4 2018 – a windy day – as well as the electric power production if sufficient wind capacity were installed in the region to supply 20% and 30% of the annually consumed electric energy in the region (in addition to the wind turbines that currently satisfy part of the demand in the ERCOT region). The power output of the four nuclear reactors in the State (4,985 MW) was added in the renewable power supply, because these units must operate continuously. It is observed that, at the 30% wind energy supply, the power produced by the wind turbines would be higher than the demand from midnight until 9:00 am. Figure 1b also depicts the power demand in the ERCOT region on April 16 2018 – a clear day with a great deal of sunshine– as well as the electric power production if sufficient PV panels were installed in the region to supply 20% and 30% of the annually consumed electric energy in the region. As with Figure 1a, the supply side includes the output of the four nuclear base-load units (4,985 MW). It is observed again that, when the solar irradiance generates 30% of the annual energy demand, then the generated power by the PV cells would be significantly higher than the demand between the hours 9:00 am and 5:00 pm. Supply-demand mismatch, such as the one shown in the lower part of the Figure generates the (now infamous) duck curve (Freeman et al., 2016; Michaelides, 2019a; Weber, 2016). The large-scale construction of Zero-Energy Buildings (ZEBs) – buildings that generate annually as much electric energy as they consume using PV cells and rely on the electricity grid for all shortfalls in the nighttime – has the same effect as the one depicted in Figure 1b. Grid-Independent Buildings (GIBs) avoid this problem by storing the surplus energy to be used when solar irradiance is not available (Leonard & Michaelides, 2018).
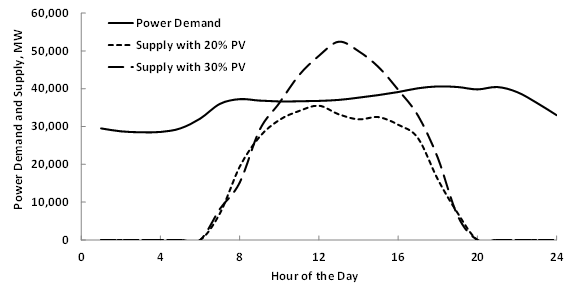
When the electric power demand is higher than the supply, the deficit is currently generated by intermediate- and peak-power units. However, when supply exceeds demand, the excess electric power in the grid must be either dissipated (and wasted) or stored to be used during another time period (El-Wakil, 1984; Leonard et al., 2020; Weber, 2016). In order to avoid the supply-demand mismatch the authorities in charge of the electricity grid may pursue a combination of the following:
-
Offer to the consumers incentives to control and adjust the electric power demand – a very difficult task to accomplish in a market-oriented economy.
-
Reduce the number of base-load units (primarily coal and nuclear) or completely eliminate them in favor of units that may be switched on and off rapidly. This entails significant investment in peak power units. In addition, the peak-power units work on natural gas or diesel and produce CO2.
-
Install utility-level storage capacity. This may also include chilled water storage that supplies the a/c demand of buildings.
All three options necessitate significant investment in equipment by the electricity production and distribution corporations, a cost that will eventually be passed to the consumers and will increase the cost of the electric power.
Energy and Sustainability Goals
Within the broad guidelines of sustainable development of the 1987 report by the U.N. World Commission on Environment and Development (Brundtland ,1987), the U.N. General Assembly approved in 2015 the Sustainable Development Goals (SDGs), a set of seventeen statements/goals that may be used for the drafting of development plans and policies in the near and intermediate future (Rosen, 2019). While all the goals are interconnected and one may see the relevance of energy availability in all of the goals, two of them are directly connected and affect the global electric power generation industry:
-
Ensure access to affordable, reliable, sustainable, and modern energy for all.
-
Take urgent action to combat climate change and its impacts.
Since energy availability and consumption are determinants of the economic development of states and regions, two additional goals are indirectly related to the affordable energy supply:
-
End poverty in all its forms everywhere.
-
Reduce inequality within and among countries.
Because climate change is inextricably connected to the production of CO2 in combustion processes, it is rational to conclude that the first two SDGs endorse the production of energy from non-fossil fuel resources, primarily from renewable sources. This will include the substitution of coal (firstly) and natural gas (secondly) in electricity generating power plants, which are point sources of CO2 emissions and currently account for approximately 40% of the global CO2 emissions.
The substitution of fossil fuel power plants with renewable sources does not happen on a one-to-one basis and depends on the type of the renewable energy source. Hydroelectric energy is the best alternative for this substitution, because hydrostatic energy is essentially stored in the water reservoirs to be used – almost at will – when the demand arises. Countries such as Norway and Canada, with a great deal of untapped hydroelectric potential, are in a very good position to substitute fossil fuels with renewable hydropower for the generation of electricity. However, wind is an intermittent energy source and the sun is a periodically variable source. Wind turbines are at standstill on calm days and solar cells do not produce any power in the nighttime. For the reliable supply of electric power, energy must be stored to satisfy the demand on calm evenings and nights, when the generation of electric power by wind and solar is almost zero.
The storage and subsequent re-generation of electricity entails thermodynamic irreversibilities. Significantly more energy must be generated by wind and solar energy to be stored and satisfy the demand on windless nights. The energy storage systems are built with additional capital expenses that, eventually, will be reflected in the cost of electricity. One of the aims of this paper is the calculation of the additional energy production and the needed energy storage requirements for the substitution of the coal and natural gas power plants within the ERCOT region. As most of the other regions in the OECD countries, the ERCOT region does not possess significant additional hydroelectric resources – beyond the currently utilized 186 MW capacity that generates 0.2% of the electricity for the grid – and must rely on solar and wind power for the substitution of the fossil fuel units.
Power Generation and Demand in the ERCOT system
Figure 2 depicts the electric power demand in ERCOT during three typical workdays of the year 2018: January 17, March 21 and July 11. The effect of the a/c demand in the summer is evident in this Figure where the daily peak power increases from 41,000 MW during the winter day and 38,000 MW during the day in the spring to more than 66,000 MW in the summer. The small difference between the winter and spring days is primarily due to the auxiliary equipment (fans and blowers) that operate with the natural gas furnaces, which provide heating to most of the buildings in the area. A salient feature of Figure 2 is that the maximum power demand in the summer occurs late in the afternoon, when the sun is setting and solar irradiance is far from its maximum. The Figure also shows that the variability of the electric power is significantly higher in the summer than in the spring and the winter: the ratio of the daily maximum to the daily minimum power demand is 1.27 for the day in January; 1.34 for the day in March and 1.77 for the day in July.
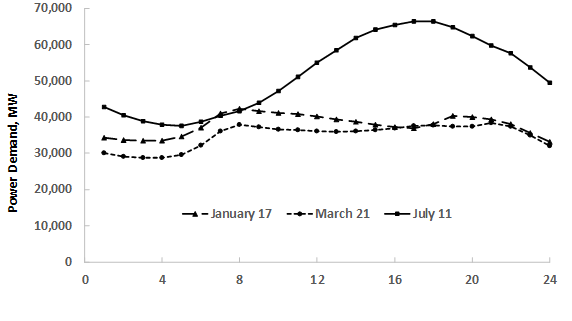
The grid is supplied by a large number of electric power generating units of all types including: nuclear, coal, natural gas, biomass, wind, solar PV, and hydroelectric. Figure 3 shows the primary energy sources that generated the annual electric energy in the ERCOT interconnect during the period 2006-2018. It is observed that natural gas and coal supplied most of the electric energy for the grid during this period. In the last year, 2018, the contribution of these two sources was approximately 69.3% of the total generated electricity, with coal supplying 24.5% and natural gas 44.8% (ERCOT, 2018). The “other” energy sources include PV solar, which currently supplies less than 0.6% of the total annual energy. It is apparent that, at present, solar energy does not significantly contribute to the production of electricity in the grid. On the contrary, wind power produced approximately 18.6% of the total electric energy in 2018; it has surpassed the generation from nuclear energy and it is projected to surpass the coal-generated electricity by 2024. The maximum electric power demand during 2018 in the grid was 73,308 MW (on July 19) and the minimum demand 27,139 MW (on April 23) (ERCOT, 2018). It is estimated (Michaelides, 2019a) that the total CO2 emissions in 2018 from all the coal power plants was approximately 107 million tons; and from the gas units and additional 125 million tons.
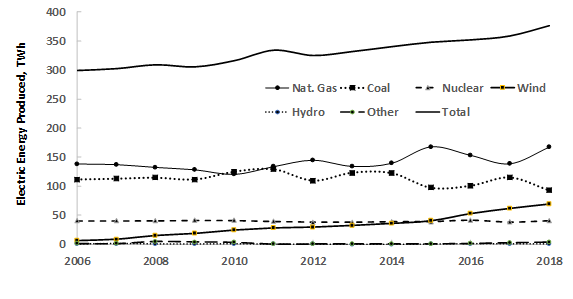
It must be noted that the total installed capacity for wind generation in ERCOT at the end of 2018 was 22,607 MW, while that of nuclear units was 4,985 MW. The latter generated power almost continuously and accounted for 41.125 TWh with aggregate capacity factor 94.5%, while the wind generators that rely on the intermittent wind power generated 69.796 TWh with aggregate capacity factor 35.2% (ERCOT, 2018).
Substitution of Fossil Fuels with Renewables
CO2 emissions by coal power plants amount to approximately 1.15 kg per kWh of generated electric energy, the highest emissions of all fossil fuel power plants. The corresponding emissions for natural gas units are approximately 0.75 kg of CO2 per kWh generated. Both numbers depend on the composition of the coal or natural gas as well as the thermal efficiency of the unit (Michaelides, 2018). Given the contributions of CO2 to GCC, and because coal units emit the highest amount of CO2 per kWh, it is sensible for electricity grids to first substitute the coal units in favor of renewables and other forms of primary energy (e.g. nuclear) that do not contribute to the GCC. Following the retirement of the coal power plants it would be advisable to retire the units that burn other fossil fuels – the natural gas units and the diesel-engine units.
The data of Figures 1 and 2 shows that this substitution is not simply achieved by constructing more solar and wind farms. When the contribution of wind and solar energy exceeds approximately 25% of the annual energy production, there are several time periods during a year, when the power produced by the renewable sources exceeds the demand. In such cases, a number of the other power plants must be shut down or the excess electric power must be dissipated and wasted. This limitation becomes particularly acute during windy weather when the wind power generation is at its peak – often during the early morning hours when the electricity demand is close to its daily minimum level. During 2016 the ERCOT grid recorded 74 hours when the spot prices of wind-generated electricity were negative because of excess wind power supply.
Shutting down base-load steam units that operate on the Rankine cycle for a few hours during a day is unrealistic, because the units need several hours to come back online. This becomes an insurmountable problem for the nuclear power plants in the USA, which utilize boron shimming for the control of nuclear reactivity and thermal power. The larger coal-fired units also require 4-6 hours to start from cold, but their output may be reduced by about 20% via steam throttling. Such time lags are high enough to preclude the Ranking cycle units from closely following the diurnal electric power demand. For this reason, the base-load power plants operate continuously, at fairly constant power levels. A glance at the data of electric power demand proves that, if the numbers of wind and solar energy units increase to produce an additional 25% of the total annual energy in the ERCOT region, the entire electric power generation system must be modified to maintain its flexibility and reliability.
A solution to this problem is to replace the inflexible steam power plants (coal and nuclear) with gas turbines and diesel engines that are flexible enough to follow the diurnal variability of the electric power demand and the production of the renewable energy units. However, this option has significant disadvantages:
-
It excludes generation from the nuclear units that currently produce very cheap electricity without any CO2 emissions and do not contribute to the GCC problem. This option also eliminates the construction of new zero-CO2 emitting nuclear power plants.
-
The natural gas and diesel power plants are fossil fuel units and emit CO2.
A second option is to develop storage systems throughout the region: the systems store the excess energy generated by the renewable sources and use this energy at a later time. The option may be extended from the coal units’ substitution to the other fossil fuel power generation units in order to achieve totally carbon-free electric power generation. This option has the additional beneficial effect of eliminating the inefficient peak-power units – older gas turbines with very low efficiency and capacity factors –that are only used for short, high-demand time periods.
The following energy storage systems are practicable for the large-scale energy storage, which is necessary for the ERCOT grid:
-
Pumped Hydroelectric Systems (PHSs) (Michaelides, 2018; Weber, 2016).
-
Hydrogen supplied to fuel cells, which are Direct Energy Conversion devices and are not subjected to the Carnot limitations (Bockris, 2002; Michaelides, 2012; Weber, 2016).
-
Chemical batteries (Batteries, 2017; Michaelides, 2018; Weber, 2016).
PHS storage is not a feasible option in the ERCOT region, because the entire region is relatively flat and does not have the river valleys and high-altitude water reservoirs that are necessary for PHSs. Chemical storage in batteries and hydrogen is feasible and the storage systems may be distributed throughout the region, with small districts and groups of buildings developing shared capacity.
It is noted that the specific energies of lithium batteries, lead batteries, and hydrogen are approximately 0.4 kWh/kg, 0.03 kWh/kg and 31.2 kWh/kg (Batteries, 2017; Michaelides, 2018). Battery storage systems are by far more massive than hydrogen systems. For short term storage (e.g. the diurnal cycle of 24 hours) solid-state batteries have higher round trip efficiencies than the hydrogen-fuel-cell combination storage. However, all batteries suffer from internal power drift (they get discharged) in the long term and this makes them unsuitable for seasonal (e.g. from spring when winds are high, to summer) energy storage. This leaves hydrogen as the most suitable energy storage medium in the region.
It must be noted that the technology for hydrogen utilization as an energy carrier is sufficiently advanced for the hydrogen storage systems to be applied to larger regions: The electrolysis processes for the production of hydrogen is simple, its technology is advanced, and the method has been used for more than two centuries. Hydrogen fuel cell technology – albeit not as advanced as that of electrolysis – has also been used for a long time and is currently used in urban transportation. In the first two decades of the 21st century, several commercial automotive corporations manufacture hydrogen fuel cell cars and buses that utilize hydrogen as a clean fuel in storage tanks at pressures up to 700 bar (Michaelides, 2018).
The System and Governing Equations
Figure 4 is the schematic diagram of the main components of a statewide system that would generate, store, and distribute renewable electric power in ERCOT. A large number of PV systems (solar farms) and wind turbines throughout the State ystemsle energy generation system generate electric power from solar irradiance and the wind. When there is sufficient demand to absorb the renewable energy, the generated power is directly fed to the grid. When the demand is low, part of the energy is supplied to the grid and the rest is diverted to water electrolysis systems that generate hydrogen gas, which is locally stored in hydrogen tanks under pressure. During periods of high power demand, the chemical energy of hydrogen is converted to electricity in fuel cells and is fed back to the grid via dc-to-ac power inverters.
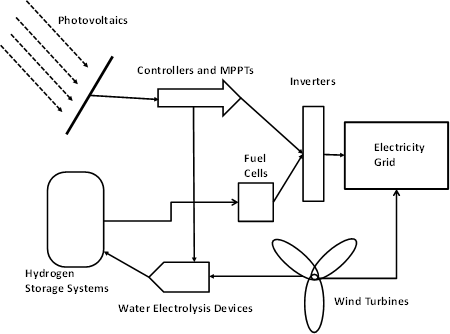
For the calculation of the parameters that would enable generation from renewables and storage in the ERCOT region, an hour-by-hour simulation was performed using the 2018 electric power demand data. Since wind and solar are the main renewable energy sources in the region, the total renewable power generation during the hour of the year, i, is the sum of the power generated by the wind and the solar installations:
\[E_{\text{Pi}} = E_{\text{WPi}} + E_{\text{SPi}}\] | (1) |
At any hour, i, the power to be stored or retrieved from storage is equal to the difference between generation and demand:
\[\delta E_{\text{Si}} = E_{\text{Pi}} - E_{\text{Di}}\] | (2) |
Because of thermodynamic irreversibilities in the energy conversion processes, part of the electric energy produced and diverted to storage is lost and is not converted to the chemical energy of hydrogen. On the other end of the storage process, part of the chemical energy of hydrogen is lost during its conversion to electricity. These energy losses, which are significant in all energy storage systems, are taken into account by the efficiencies of the electrolytic process, ηel, and of the fuel cells, ηfc. Accordingly, the stored energy (energy storage level) in the (i+1)st hour becomes:
\[\begin{matrix} E_{Si + 1} = E_{\text{Si}} + \left( \delta E_{\text{Si}} \right)\eta_{\text{el}}\text{ if }E_{\text{Pi}} \geq E_{\text{Di}} \\ E_{Si + i} = E_{\text{Si}} - \left( \delta E_{\text{Si}} \right)/\eta_{\text{fc}}\text{ if }E_{\text{Pi}} < E_{\text{Di}} \\ \end{matrix}\] | (3) |
where ESi is the energy storage level at the previous hour, i. The values of the efficiencies used in this study are: \(\eta_{\text{el}}\) = 78% (Mazloomi et al., 2012), and \(\eta_{\text{fc}}\) = 75% (US-DOE Hydrogen Fuel Cell factsheet, 2006). Small amounts of energy are also dissipated in the other equipment of Figure 4: the efficiencies of the Maximum Power Point Trackers (MPPTs) are higher than 95% (Haeberlin et al. 2006, 2017); modern dc to ac inverters also exhibit efficiencies that are higher than 95%. For the calculations these efficiencies are lumped with the efficiencies of electrolysis and of the fuel cells.
The entire generation-storage system must be robust and flexible enough to satisfy the diurnal, seasonal, and annual power demand fluctuations, which become higher during the summer months, when the a/c demand is high in the entire region served by ERCOT. For the reliability of the entire grid system, when all fossil fuel power plants are substituted by renewables, it was stipulated that the energy storage tanks must always have enough capacity to supply the entire grid for a minimum of ten days. If there is a system failure, malfunction or adverse weather conditions that temporarily reduce the amount of renewable energy produced, then the grid operators will have ample time to purchase hydrogen, or divert energy from a different geographical area to ensure that the electricity supply in the grid will continue uninterrupted. As a result of this constraint the stored energy in the entire system is significantly higher than zero during all hours of the year.
Storage and Additional Energy Needs
Calculations were first performed for the substitution of the coal power plants in the ERCOT grid system, which produce significantly more CO2 (and other pollutants) per kWh generated than the gas turbines, and are widely considered to be the first power units to be substituted. Simulations were performed for every hour of the year 2018, using the power demand data of ERCOT (Ercot, n.d.). In 2018 the several coal power plants within the area supplied by the grid produced 93.249 TWh of electricity (24.8% of the total generation). The calculations included several combinations of wind and solar generation and the results are depicted in Figure 5. The ordinate of the figure is the percentage of wind (100% minus the ordinate value is the percentage of solar) power used for the substitution of the coal power plants. The two abscissas of the figure show: on the right the storage capacity, in m3, that is necessary for the substitution at the given power mix of wind and solar; and on the left the electric energy, in MWh, that must be produced by the PV installations and the wind installations, as well as the energy lost during the storage and re-generation processes. It is observed that minimum storage capacity is required (and also minimum energy is lost in the storage-regeneration processes) when the additional renewable energy is generated in approximately equal parts from solar and from wind. The flat energy loss curve denotes that minimum energy losses also occur when the mix of the additional wind and solar energy are approximately equal. Figure 5 also shows that, if all the additional energy is generated by the wind – by continuing the current trends of adding wind farms throughout Texas, – then 254.4 million MWh must be produced by the new wind farms (of which 89.7 million MWh would be lost in the storage-regeneration processes) and a storage capacity of 10.3 million m3 must be built.
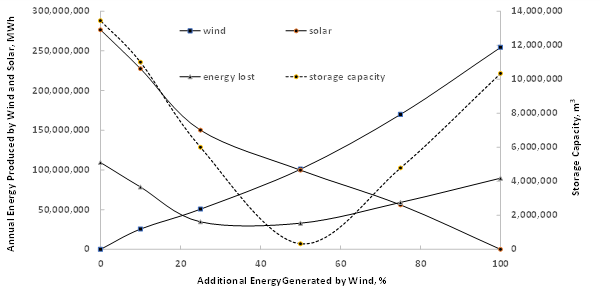
The second set of calculations was performed for the substitution of all the fossil fuel powered units in ERCOT, coal as well as natural gas power plants. The coal power plants produced approximately 24.8% of the electricity demand in 2018 and natural gas turbines produced an additional 44.5%. Figure 6 depicts the storage requirements for this substitution; the additional energy that must be generated from wind and solar; and the annual energy lost in the storage-regeneration system. It is observed that, for the entire substitution of the fossil fuel units, the minimum storage capacity (29.8 million m3) occurs when only 20% of the additional renewable energy is generated from wind and the remaining 80% from solar. This happens because energy generated from solar installations is better correlated with the diurnal power demand, and especially with the power peaks on summer days that are due to a/c usage. The minimum energy lost (31.5 million MWh) corresponds to approximately 75% wind power and 25% solar power mix for the additional energy that needs to be produced annually. With this mix of renewable energy generation, the required storage capacity is 41.7 million m3.
Table 2 gives the additional power of PV and wind turbines that (together with the storage system) would generate the additional energy to substitute the coal power plants and all the fossil fuel plants. In the latter case the minima of lost energy and storage capacity do not coincide, as it is evident in Figure 6. The data for the 100% substitution by wind are offered because, at present, the generation of power by wind turbines is by far less expensive than generation by PV cells, even when the governmental subsidies are taken into account. As a consequence, there is a great deal of investment and strong growth in the wind power installed capacity.
Table 2. Additional renewable power needs for the substitution of fossil-fueled power plants
|
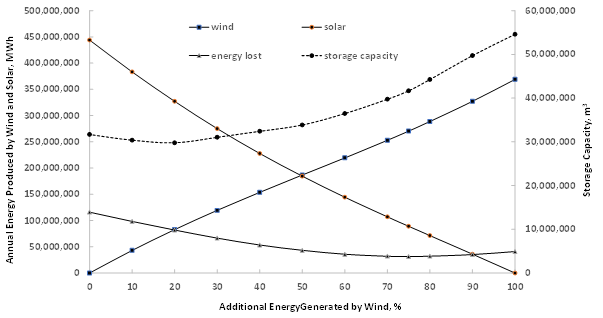
It must be noted that, because the two renewable energy sources do not produce power continuously and because of the energy lost in the storage-regeneration process, significantly more solar and wind capacity must be installed than the substituted fossil fuel units. For example, the 80,700 MW combined installed capacity in the first line of Table 2 would substitute approximately 14,000 MW of coal-fired units. Because of the low energy generation capacity factors (approximately 36% for wind and 22% for solar) the substitution of fossil fueled units with renewable units does not happen on one-to-one basis of rated capacity. The additional installed capacity together with the installation costs of the storage systems would add to the price of electricity and will, eventually, be passed to the consumers.
Addition of Nuclear Generating Capacity
A glance at Table 2 proves that the required storage capacity and the combined wind and solar power installations are very high and this would require substantial capital investment. A viable method to significantly decrease the installed renewable power and the storage capacity is to increase the energy generation contribution of nuclear power within the ERCOT grid by constructing new nuclear power plants, which do not emit CO2. Since the nuclear power plants are base-load units, effectively the construction of more nuclear reactors would balance the decommissioning of the coal power plants – also base-load units. Table 3 shows some of the results for the substitution of all fossil fuel power plants with wind and solar renewable sources when the installed nuclear capacity is 4,985 MW (the current capacity); 9,970 MW (twice the current capacity); and 19,940 MW (four times the current capacity).
Table 3. Additional renewable power needs and storage capacity when the nuclear capacity is doubled and quadrupled
|
It is observed in Table 3 that both the storage requirements and the renewables installed capacity decrease significantly when the nuclear units are increased. Because the nuclear power plants are base-load plants, the additional nuclear capacity is significantly lower than the capacity of the renewable sources they would substitute. It may be seen in Table 3 that the addition of 4,985 nuclear capacity alleviates the addition of 16,500 MW of wind and solar combined installed capacity at the minimum energy lost option; and 17,100 MW of wind capacity at the 100% wind option.
Effects on Prices, Incomes and Sustainability Goals
Prices and costs are estimated using the theories of economics, which is a social science based on empirical principles and not natural laws. Unlike the principles of thermodynamics that are natural laws, the principles of economics emanate from correlations of empirical data; they are not permanent; and they heavily depend on the time of observations and the type of economy they were made – e.g. the energy price mechanisms are different in free-market economies, regulated economies, and centrally-planned economies. All energy prices (including the cost of power installations) exhibit high variability over long time periods, and are laden with significant uncertainty (Michaelides, 2017, 2018). For this reason, future prices and costs should be viewed as estimates with significant variability, rather than accurate calculations. A second source of variability of the renewable energy units is the regional variation of solar irradiance and wind velocity: because of the lower irradiance in the northern regions, the same PV cells would produce only 54% of the annual energy in Duluth Minnesota, than in Dallas, Texas. The lesser energy production from the same (and equally priced) PV systems would be reflected in higher prices of electricity, expressed as $/kWh.
A complication that often enters price calculations is that, at present, renewable energy is subsidized by the governments. However, when renewables produce a high fraction of the electricity in a region the subsidies expire. As a result, the current price of electricity production from renewables may not reflect the price after all the subsidies expire. Wind power is currently subsidized in Texas by $23/MWh for the first ten years of power production by the wind farms. New wind farm subsidies in ERCOT will not be available in 2020, because the technology is considered mature to survive on its own. Both solar and wind power production tax credits and subsidies will be phased out by 2025 (Rhodes, 2019) unless extended by a new law. Similarly, the renewable energy subsidies will be phased out in other countries when solar and wind power generate a sizable fraction of the electricity. On the opposite end of power generation, several studies that calculate the cost of electricity from coal and natural gas include a cost of carbon abatement or the cost for carbon capture and sequestration, environmental costs for the cleanup of CO2, and other pollution products (Lazard, 2016). Since, at least at the present time, these costs are not born by the electricity generation corporations in any form, they will not be considered in this study.
The average retail price of electric energy paid by the residential sector in the ERCOT region in 2019 is $0.108/kWh including all applicable taxes (tcap, 2018). Of this, approximately $0.05/kWh accounts for the energy transmission cost, which is born by the consumers. The prices for the commercial and industrial sectors are significantly lower, with the average of all sectors being $0.086/kWh (tcap, 2018). Because the ERCOT region is “deregulated,” several competing corporations offer their own plans to residential customers. The retail prices in these plans depend on the specific area, where electricity is consumed: in the Houston area the retail residential prices are in the range $0.089-$0.150, while in the Dallas-Fort Worth Metroplex the retail residential prices are in the $0.079-$0.140 range (tcap, 2018). These residential prices are lower than the average USA electricity prices and significantly lower than the retail residential prices in most of the industrialized countries in the world (International Energy Agency, 2019). The low electricity prices have helped residents afford a/c systems that are now necessary in all households and businesses.
A glance at the previous sections proves that the substitution of fossil fuel power plants with renewables will entail the following expenses, which in a market economy would be passed to the consumers:
-
Cost of construction of the renewable installations (e.g. wind and solar farms). As it becomes apparent from Tables 2 and 3, the installed capacity of renewables is 3-5 times higher than the capacity of the units they substitute.
-
Cost of the energy storage systems and of the energy lost in the storage-regeneration process. Several sources of the life-cycle costs of energy storage systems were assessed and compared in Zakeri and Syri (2015). It becomes apparent in this and similar studies that storage costs will add significantly to the price for electricity paid by the consumers, even if the energy storage is accomplished in electric vehicle batteries (Michaelides, 2019b).
Now, let us assume that the substitution of the fossil fueled power plants becomes a reality. This will trigger the following effects:
-
The CO2 emissions in the electricity generation sector of Texas (and also of the entire USA) will plummet, a desirable effect.
-
The amount of the generated electric energy will increase because of the irreversibilities in the storage-regeneration process. This effect is not desirable, but it can be accommodated by the society at large.
-
The price of electricity in the region will increase, very likely significantly because of items A and B, above. With the nuclear capacity unchanged; with all fossil fuel generating plants decommissioned; and with the estimated costs for solar and wind power generation without subsidies (Ferreira et al., 2018; Hoffmann, 2006; Zakeri & Syri, 2015) and including storage and transmission, the estimates of the future electricity price for the consumers are in the range $0.30/kWh-$0.45/kWh, a significant increase over the current electricity prices.
The sharp increase of electricity prices – by a factor between 2.8 and 4.2 – will have a significant effect on the population that would have to pay more for their electricity consumption, in particular during the hot summer months. Residents in the low income brackets may not be able to afford complete a/c services for their households and this may become a health hazard, especially for the older and the infirm; residents in the intermediate income brackets will spend a significantly higher fraction of their income on electricity and will have less disposable income for other necessities or pleasure activities; high-income residents will be able to afford the electricity price increases albeit at a reduction of their disposable income. The net result of these expected effects is an increase in the disposable income inequality of citizens in the region and this is incongruent with the goal of reducing inequality within and among the nations – the 4th goal in the section on “Energy and Sustainability Goals.” A projection of the expected increase of electricity prices to other nations, also leads to the conclusion that, with a fully renewable energy economy, it will become more expensive for citizens of less affluent nations to enjoy the fruits of a/c at home and higher mechanization at work. As a consequence, the goal of reducing poverty in all its forms may suffer. An additional effect on the economy of nations is that, as a result of the reduction of the disposable income of a large fraction of the population, other economic sectors will suffer and national economies as a whole will shrink (Samuelson & Nordhaus, 2009).
The higher prices of electricity may lead to a reduction of its demand, which would partly alleviate increased prices. If prices increase significantly, the demand by the consumers may decrease. However, one must take into account that, for most citizens, electricity is a necessity and its price elasticity is very low. Most energy-saving projects – e.g. increasing the buildings’ insulation and installation of more efficient a/c systems – are capital intensive and are not readily affordable by citizens in the lower income brackets. As societies move toward a “greener” mix for the production of electricity, they must ensure that low-interest loans and other subsidies become available to low-income homeowners for energy conservation measures.
The correlation of higher electricity prices and income inequality is similar to the effect of a carbon tax on the incomes of the citizens. Two recent studies (Goulder et al., 2019; Williams III et al., 2015) determined the effects of a carbon tax on the incomes of USA citizens in five income categories when all the tax is returned (recycled) to the citizenry: as lump-sum payments to the lower incomes; as capital tax relief; and as labor tax relief. Table 4 shows these effects for the five income quintiles (Williams et al., 2015) with the 1st quintile being at the lowest income.
Table 4. Effect of a carbon tax with three kinds of tax recycling in the USA ( Williams III et al., 2015)
|
It is observed in Table 4 that if the carbon tax is not recycled back to the citizenry with direct payments to the lowest incomes, the increase of the energy prices will have a significant and negative effect on the lower income citizens. The same conclusion was reached in Goulder et al. (2019), whose authors determined that anything other than lump-sum rebates to the lower income citizens would constitute a regressive taxation. Clearly, the substitution of fossil fuels with renewables or a carbon avoidance tax, which is designed to promote the substitution of fossil fuels with renewables, must be accompanied by public policy measures that would protect the incomes and the lifestyles of the poorer citizens in a society.
CONCLUSIONS
The substitution of coal and natural gas electric power plants with renewable energy is desirable for the avoidance of GCC. However, and because solar energy is periodically variable and wind power is intermittent, this substitution will not be realized without the development of infrastructure for the storage of energy. The substitution of, firstly coal and, secondly, natural gas from the electricity production mix in the ERCOT electricity grid is feasible as an engineering project, but entails the installation of significantly higher wind and PV capacity; high energy storage capacity; and significant energy losses in the storage-recovery process. Given the terrain of the region, hydrogen storage is the most suitable energy storage method, with hydrogen produced by electrolysis, stored locally in tanks and converted to electricity with fuel cells. The addition of more nuclear capacity is beneficial because it reduces significantly the requirements for storage and the additional installed capacity.
The installation of significantly higher generating capacity, the development of energy storage systems, and the thermodynamic irreversibilities associated with the energy storage and recovery processes, are very likely to increase significantly the price of electricity for the consumers. This is an undesirable outcome that affects disproportionately the less affluent members of the society and is incongruent with the goals to reduce poverty and reduce inequalities. It would be advisable that attention be given to this undesirable outcome by governments, which may adopt public policies, price structures, and subsidies that that would make electricity affordable for all.
ACKNOWLEDGEMENTS
This research was partly supported by the Tex Moncrief Chair of Engineering at TCU.
-
Hydroelectric power plants may be used either as base-load or intermediate-load units.↩
-
ERCOT is the Electric Reliability Council of Texas, which manages the production and distribution of electric power and supplies electric power to 92% of the State’s residents. The entire annual electricity generation in this grid is higher than those of the UK and Italy.↩
References
- Batteries. (2017). North America Clean Energy, 10(3), 40-45.
- Bockris, J. O’M. (2002). The Origin of Ideas on a Hydrogen Economy and Its Solution to the Decay of the Environment. Intern. J. of Hydrogen Energy, 27,731-740. https://doi.org/10.1016/S0360-3199(01)00154-9
- Boden, T. A., Marland, G., & Andres, R. J. (2012). Global, Regional, and National CO2 Emissions, Trends: A Compendium of Data on Global Change, ORNL Report, Carbon Dioxide Information Analysis Center, Oak Ridge, TN, USA.
- Brundtland, G. H. (1987). Report of the World Commission on Environment and Development: Our Common Future. Retrieved on 20 September 2019 from http://www.un-documents.net/our-common-future.pdf
- El-Wakil, M. M. (1984). Power Plant Technology. New York: McGraw Hill.
- ERCOT. (2018). Monthly Demand and Energy Report. Austin, TX, USA: ERCOT Inc. Report.
- Ercot. (n.d.). Generation. Retrieved on 20 September 2019 from http://www.ercot.com/gridinfo/generation
- Ferreira, A., Kunh, S. S., Fagnania, K. C., De Souza, T. A., Rodrigues Dos Santos, C., & Coimbra-Araújo, C. H. (2018). Economic overview of the use and production of photovoltaic solar energy in Brazil. Renewable and Sustainable Energy Reviews, 81, 181-191. https://doi.org/10.1016/j.rser.2017.06.102
- Freeman, E., Occello, D., & Barnes, F. (2016). Energy Storage for Electrical Systems in the USA. AIMS Energy, 4, 856-875. https://doi.org/10.3934/energy.2016.6.856
- Goulder, L. H., Hafstead, M. A. C., Kim, G. R., & Long, X. (2019). Impacts of a carbon tax across US household income groups: What are the equity-efficiency trade-offs? J. Public Economics, 175, 44-64. https://doi.org/10.1016/j.jpubeco.2019.04.002
- Haeberlin, H., Borgna, L., Kaempfer, M., & Zwahlen, U. (2006). Measurement of dynamic MPP-tracking efficiency at grid-connected PV inverters. 21st European Photovoltaic Solar Energy Conference and Exhibition, Dresden.
- Hoffmann, W. (2006). PV solar electricity industry: market growth and perspective. Sol Energy Mater Sol Cells, 90, 3285-3311. https://doi.org/10.1016/j.solmat.2005.09.022
- International Energy Agency. (2003). Key World Statistics-2003, IEA-Chirat, Paris.
- International Energy Agency. (2018). Key World Statistics-2018, IEA-Chirat, Paris.
- International Energy Agency. (2019). Electricity Information. France: IEA Publications. https://doi.org/10.1787/e0ebb7e9-en
- Lazard (2016). Lazard’s levelized cost of energy analysis – version10.0. Paris.
- Leonard, M. D, & Michaelides, E. E. (2018). Grid-Independent Residential Buildings with Renewable Energy Sources, Energy, 148C, 448-460. https://doi.org/10.1016/j.energy.2018.01.168
- Leonard, M. D., Michaelides, E. E., & Michaelides, D. N. (2020). Energy storage needs for the substitution of fossil fuel power plants with renewables. Renewable Energy, 145, 951-962. https://doi.org/10.1016/j.renene.2019.06.066
- Mazloomi, K., Sulaiman, N., & Moayedi, H. (2012). Review - Electrical Efficiency of Electrolytic Hydrogen Production. Int. J. Electrochem. Sci., 7, 3314-3326.
- Michaelides, E. E. (2012). Alternative Energy Sources. Berlin: Springer. https://doi.org/10.1007/978-3-642-20951-2
- Michaelides, E. E. (2016). Future Directions and Cycles for Electricity Production from Geothermal Resources. Energy Conversion and Management, 107, 3-9. https://doi.org/10.1016/j.enconman.2015.07.057
- Michaelides, E. E. (2017). A New Model for the Lifetime of Fossil Fuel Resources. Natural Resources Research, 26, 161-175. https://doi.org/10.1007/s11053-016-9307-2
- Michaelides, E. E. (2018). Energy, the Environment, and Sustainability, CRC Press, Boca Raton. https://doi.org/10.1201/b22169
- Michaelides, E. E. (2019a). Making Texas Green. Mechanical Engineering, 141(3), 32-37. https://doi.org/10.1115/1.2019-MAR-2
- Michaelides, E. E. (2019b). Thermodynamics and Energy Consumption of Electric Vehicles. Energy Conversion and Management. https://doi.org/10.1016/j.enconman.2019.112246
- Rhodes, J. D. (2019). Tax Credits for Wind and Solar Work. Why are Texas Lawmakers Going After Them? Texas Perspectives, April 12.
- Rosen, M. A. (2019). Advances in Sustainable Development Research (editorial). Europ. J. of Sustainable Development Research, 3(2), em0085. https://doi.org/10.29333/ejosdr/5730
- Samuelson, P. A., & Nordhaus, W. D. (2009). Economics, (19th Edition). New York: McGraw-Hill Education.
- tcap (2018). Electricity prices in Texas, 2018. Retrieved on 15 October 2019 from www.tcaptx.com/reports
- US-DOE Hydrogen Fuel Cell factsheet, DOE Hydrogen program, October 2006.
- US-Energy Information Administration. (2016). International Energy Statistics – Electricity, Washington DC.
- Weber, M. E. (2016). Making Renewables Work. Mechanical Engineering Magazine – ASME, 138(12), 12.
- Williams III, R. C., Burtraw, D., & Morgenstern, R. D. (2015). The Impacts of a US Carbon Tax across Income Groups and States. Resources, 56, 25-29.
- Worland, J. (2018). The chilling impact of air-conditioning, Time Magazine, July 30.
- Zakeri, B., & Syri, S. (2015). Electrical energy storage systems: A comparative life cycle cost analysis. Renewable and Sustainable Energy Rev., 42, 569-596. (Corrigendum, 53, 1634-1635). https://doi.org/10.1016/j.rser.2014.10.011
How to cite this article
APA
Michaelides, E. E. (2020). Fossil Fuel Substitution with Renewables for Electricity Generation – Effects on Sustainability Goals. European Journal of Sustainable Development Research, 4(1), em0111. https://doi.org/10.29333/ejosdr/6344
Vancouver
Michaelides EE. Fossil Fuel Substitution with Renewables for Electricity Generation – Effects on Sustainability Goals. EUR J SUSTAIN DEV RES. 2020;4(1):em0111. https://doi.org/10.29333/ejosdr/6344
AMA
Michaelides EE. Fossil Fuel Substitution with Renewables for Electricity Generation – Effects on Sustainability Goals. EUR J SUSTAIN DEV RES. 2020;4(1), em0111. https://doi.org/10.29333/ejosdr/6344
Chicago
Michaelides, Efstathios E.. "Fossil Fuel Substitution with Renewables for Electricity Generation – Effects on Sustainability Goals". European Journal of Sustainable Development Research 2020 4 no. 1 (2020): em0111. https://doi.org/10.29333/ejosdr/6344
Harvard
Michaelides, E. E. (2020). Fossil Fuel Substitution with Renewables for Electricity Generation – Effects on Sustainability Goals. European Journal of Sustainable Development Research, 4(1), em0111. https://doi.org/10.29333/ejosdr/6344
MLA
Michaelides, Efstathios E. "Fossil Fuel Substitution with Renewables for Electricity Generation – Effects on Sustainability Goals". European Journal of Sustainable Development Research, vol. 4, no. 1, 2020, em0111. https://doi.org/10.29333/ejosdr/6344